1. Introduction
2. Materials and methods
2.1 Materials
2.2 Dissimilar laser welding and self-restraint test
Fig. 1

Table 2
2.3 Microstructure analysis
3. Results and discussion
3.1 Microstructures of base metal
3.2 Microstructure and solidification cracking behavior of Cu-mild steel laser welds
Fig. 3
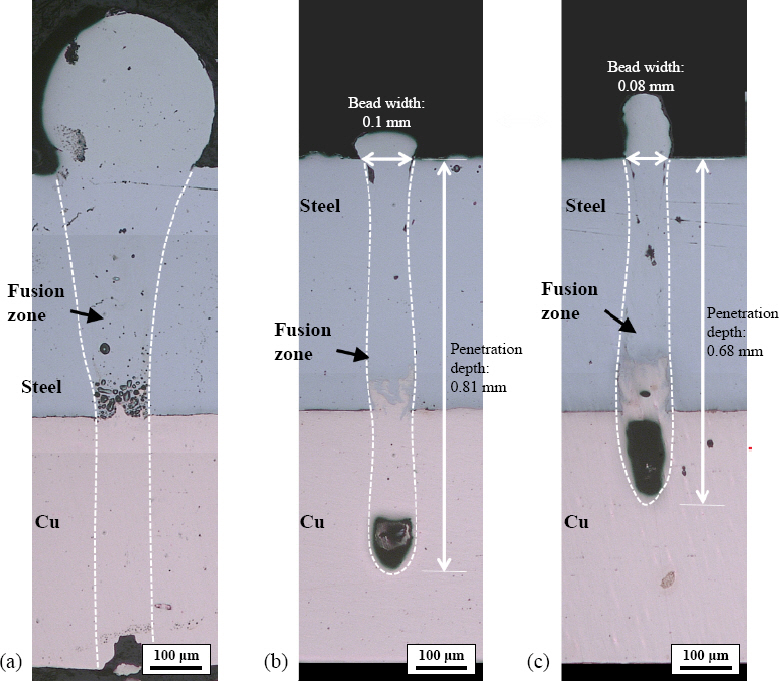
Fig. 4
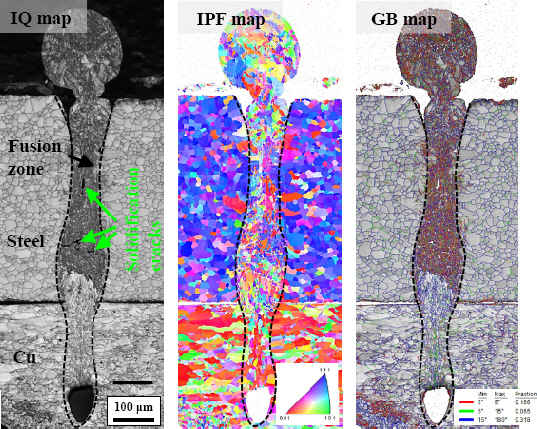
Fig. 5
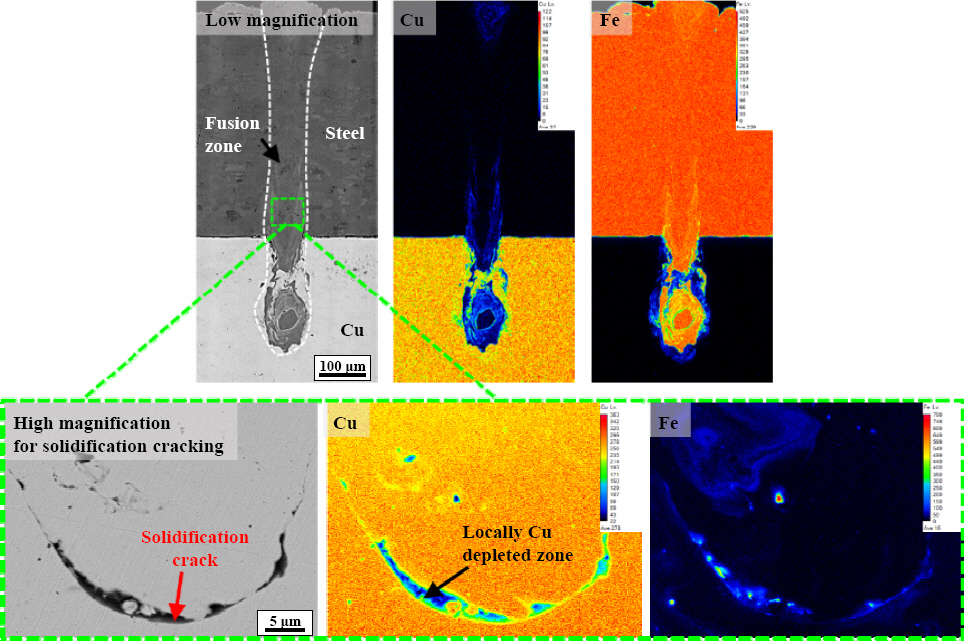
3.3 Clarification of solidification cracking mechanism at locally Cu-depleted zone by self- restraint test
Fig. 7

Fig. 8

Fig. 9
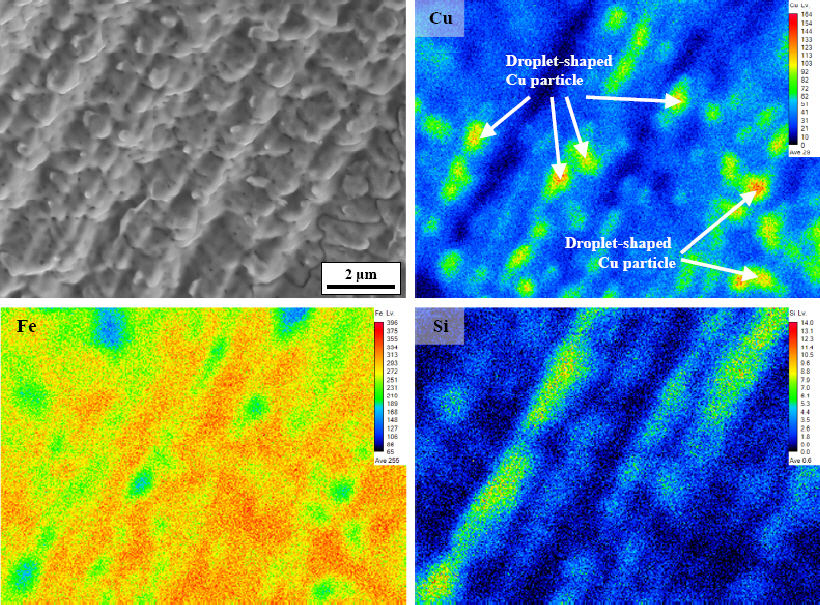
3.3.1 Estimation of non-equilibrium mushy zone range for Cu depleted zone solidification cracking
Table 3
Laser sources | Laser power (kW) | Defocus distance (mm) | Upper material | Lower material | Scan speed (mm/s) | Heat input (J/mm) | Energy density (J/mm2) |
Single-mode fiber laser | 2 kW | 10 | Steel | Cu | 250 | 8 | 260 |
Table 4
Cu mixture (mass%) | Solidification completion ratio (%) | Cooling rate (K/s) | Secondary dendrite arm spacing (㎛) |
---|---|---|---|
5mass%Cu-SS400 steel | 99 | 2000 | 1 |
15mass%Cu-SS400 steel | |||
25mass%Cu-SS400 steel |
Fig. 10

4. Conclusion
1) Under the welding conditions employed for both single-mode fiber (upper material: mild steel, lower material: Cu) and green (upper material: Cu, lower material: mild steel) laser welding processes, both materials did not mix within the fusion zone. In addition, solidification cracking occurred in the steel part of the fusion zone, particularly in the locally Cu-depleted region.
2) To clarify solidification cracking in the Cu-depleted region, a self-restraint test was performed on dissimilar materials using single-mode fiber laser welding. The analysis results of the solidification cracking surface showed that approximately from 15 to 30 mass% of Cu existed. Moreover, the Cu had a droplet shape, which is considered to be formed during the solidification at the miscibility gap.
3) By calculating the weld mushy zone range based on Scheil’s model, the solidification cracking in the Cu-depleted region was estimated at 453 K, affected by the severe segregation of Cu (95.7 mass%) in the residual liquid at the terminal stage of the solidification path.